The Universe May Be More Habitable Than We Thought
By Lynn Rothschild, Ph.D.
NASA Ames Research Center
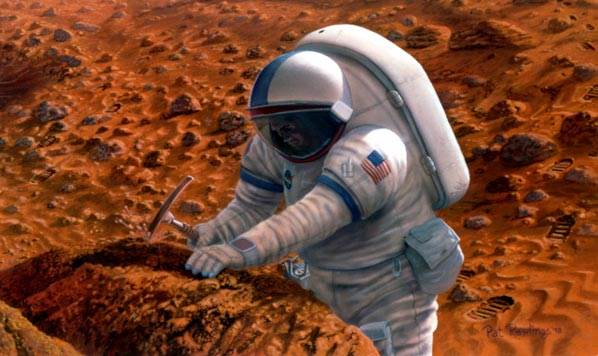
The crew of Starship Enterprise regularly boasts that they go where no one has gone before. Alas, scientists have discovered that life on Earth has already done that — and has done so for billions of years. Life flourishes in physical and chemical extremes that, until recently, were thought to preclude life — hence the term “extreme” that is often used to characterize these locales. These extreme environments — hot, cold, acidic, saturated by radiation — are also similar to what we expect to find on other worlds. As such astrobiologists view these environments and the life that flourishes there as a preview of what we might find elsewhere in the universe.
The various unique physiologies that have evolved to meet the challenges poised by these “extreme” environments demonstrate that life could exist in some of the extreme environments found in space and beyond. Indeed, adaptations have been found in some terrestrial organisms that could allow travel between planetary bodies.
Where are these extreme places? What challenges do they present to life — and how has life adapted? And what does this say about life elsewhere?
CHEMISTRY
Organic chemistry — chemistry based on reduced forms of carbon (“reduced” carbon has had hydrogen added) has been shown to operate not just upon on our planet, but across our solar system, and far, far beyond. Carbon can trump even silicon (a common component of rocky planets) in its ability to form an astonishing variety of long and complex compounds. It is these long complex molecules that make life capable of what it does. Indeed, there is quite a ubiquity of organic chemistry in the universe: many of the compounds associated with terrestrial life have been found to be floating in the vast spaces between stars.
WATER – LIFE’S SOLVENT
Water is an excellent solvent for organic molecules — it provides a context wherein increasingly complex chemical reactions can occur — and be sustained. Based on what we have seen of life, it appears that liquid water is the sine qua non of life. Based on this understanding, the official mantra of the current Mars program at NASA is to “follow the water.” Admittedly organic carbon (in contrast to carbon dioxide and carbon monoxide) has yet to be detected on Mars — but we’re looking for it!
If water is indeed essential for life, a variety of physical limits to life seem apparent. But “seems” is the operative word. What may seem to be true in a theoretical or experiential context may not be true once sufficient observations have been made.
Water is a liquid and remains so within certain physical criteria such as temperature and pressure. Too much and too little of either can bring life’s processes to a halt. As water becomes scarce, a struggle for survival ensues. For life to continue, temperature has to be within the range wherein water can exist in liquid form. We have yet to find any form of life that can directly utilize solid (i.e. frozen) water.
Temperature has another importance: organic molecules lose the structure necessary for them to function (i.e. they “denature”) at certain temperatures. For both DNA and chlorophyll (the molecule at the core of photosynthesis) this temperature is around 70°C. On the other hand, as temperature drops, biochemical reactions slow. Ice crystals which begin to form within cells which can cause irreparable harm as they slash through cellular membranes. Membranes are the surfaces upon which life’s myriad reactions occur. They also serve to contain a cell’s contents. Their damage slows down an organism’s biochemistry.
Other factors inhibit life’s ability to operate: extremes in pressure can destroy molecular structures and inhibit enzymatic reactions. Then there are toxins in the environment, such as mercury, arsenic and cadmium which can poison metabolism. High levels of radiation can damage a variety of organic molecules, most notable among these being the very genetic material of the cell, DNA. Ditto for oxygen.
MY AIR IS YOUR POISON
Wait! — oxygen as an extreme environment? Oxygen allows the production of ATP, the energy currency utilized by all cells. This process is 18 times more efficient than anaerobic metabolism, i.e. metabolism that occurs in the absence of oxygen. However, this increased efficiency comes at a steep price. The reduced (hydrogenated) forms of oxygen, such as hydrogen peroxide and especially the hydroxyl radical, may be extremely dangerous. The resulting oxidative damage they can cause can damage DNA, causing mutations or even death. Anaerobes (organisms that do not use oxygen in their metabolic processes) do not have the ability to detoxify the various forms of oxygen, and accordingly find oxygen lethal.
As such, from the perspective of a substantial portion of the life on Earth, the ability to live in an aerobic (oxygen rich) world confers upon our own species the distinction of being an extremophile. But there are other things that organisms can “breathe.” The bacterium Shewanella putrefaciens uses metal atoms in its metabolism in the same fashion as we use oxygen atoms. As such, it “breathes” metal — in this case, manganese.
Clearly there are physical and chemical extremes that should make life based on organic carbon difficult if not impossible. Yet, within the last few decades we have found organisms that have punctured these seemingly insurmountable limits and have come to called “extremophiles” from the Latin “extremus” (being on the outside) and the Greek “philos” for love. Organisms that can live in more than one extreme, for example Sulfalobus acidocaldarius, a member of the Archea (an ancient branch off the family tree of life) which lives at pH 3 and 80°C, are called “polyextremophiles.”
WHO ARE THE EXTREMOPHILES?
The word “extremophile” often invokes images of microbes, and so-called “simple” ones at that, yet the taxonomic range spans all three domains. (Note that life itself is so complex that the human creation of life has remained elusive. Thus, it is unjustifiably arrogant of us to call any form of life “simple.”) While all organisms that live at extremely high temperatures are Archaea or Bacteria, eukaryotes (organisms whose cells have nuclei) are common among organisms that thrive at low temperature, extremes of pH (high acidity or alkalinity), pressure, water, and salt levels. Extremophiles include multicellular organisms, cold-lovers include vertebrates such as penguins and polar bears.
To qualify as an extremophile, does an organism have to be an extremophile during all life stages? Under all conditions? Not at all. Spores, seeds, and sometimes eggs or larval stages are all far more resistant to environmental extremes than adult forms. Yet some adult organisms — trees, frogs, and insects — can endure remarkably low temperatures during the winter as a result of seasonal shifts in physiology such as hibernation.
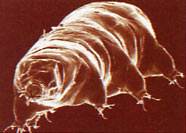
One of the most resilient organisms known are tardigrades (“water bears”). Tardigrades can go into a hibernation mode — called the tun state — one that is more akin to “suspended animation” whereby it can survive temperatures from -253°C to 151°C, as well as exposure to x-rays, and vacuum conditions. When you place tardigrades in perfluorocarbon fluid (again while hibernating), at a pressure of 600 MPa (that’s almost 6,000 times atmospheric pressure at sea level), they emerge from the experience just fine. Even the bacterium Deinococcus radiodurans, the most radiation resistant organism known, only achieves this resistance under some conditions such as fast growth and in nutrient-rich medium.
WHO? WHAT? HOW?
Living in a Goldilocks world that is not too hot, not too cold and so on is the easiest environment for life to exist. An extremophile must either live within these parameters, or guard against the outside world in order the maintain these conditions intracellularly.
DRY ENVIRONMENTS
Imagine a desert and a feeling of dehydration follows. In the absence of water, lipids (fats), proteins and nucleic acids (DNA, RNA) suffer structural damage. The Atacama desert located on the high northern Andean plains of Chile is one of the oldest, driest hot deserts on the Earth, while the Antarctic dry valleys are the coldest, driest places on Earth. In both cases, despite environmental extremes, life exists in the form of microbes: cyanobacteria, algae, lichens, and fungi.
Anhydrobiosis is a strategy organisms use to survive dry spells. During anhydrobiosis their cells come to contain only minimal amounts of water. No metabolic activity is performed. A variety of organisms can become anhydrobiotic, including bacteria, yeast, fungi, plants, insects, the aforementioned tardigrades, mycophagous (fungi-eating) nematodes, and the brine shrimp Anemia sauna (also known as “Sea Monkeys” when marketed to school age children). During the drying out process (desiccation), less available water forces substances to increase in their concentration. Such increases lead to stressful responses within a cell that are similar to those a cell experiences when exposed to high salt environments.
The ultimate dry environment is the “desert” of space. Adaptations to desiccation are critical for organisms to survive in interplanetary space. One organism in particular (described below) is a natural born space traveler.
SALINITY
As airplanes descend into the San Francisco area, red patches on the eastern shore of the South Bay are conspicuous. These are evaporation ponds of Cargill Salt Company. The cause of the red color is halophilic (salt-loving) microbes that produce red pigments called carotenoids. A similar situation occurs in salt flats, such as the Great Salt Lake in Utah, and deep sea hypersaline basins. The microbes involved are either members of the Archaea, a major group of microbes superficially similar to bacteria, or the green alga Dunaliella salina. At a bit lower (25-33% ) salinity, bacteria, cyanobacteria, other green algae, diatoms and protozoa are found. Some Archaea, cyanobacteria, and Dunaliella salina can even survive periods in saturated sodium chloride — about as salty an environment as one can imagine.
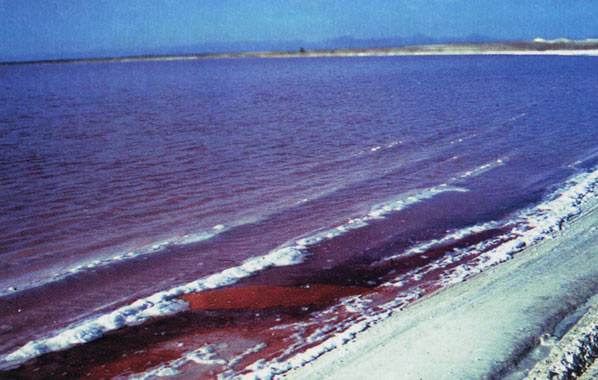
Salt water can evaporate leaving deposits (“evaporite deposits”) consisting of salts such as sodium chloride (halite) and calcium sulfate (gypsum). Within evaporates are fluid inclusions — small trapped pockets of water — which can provide a refuge for microbes for at least six months. Our research group showed that cyanobacteria trapped within dry evaporite crusts can continue to have low levels of metabolic function such as photosynthesis. These deposits also form nice fossils of the organisms trapped within. Although highly controversial, others claim that bacteria might survive for millions of years in the fluid inclusions of salt deposits including evaporates. Tantalizingly, such deposits have been found on Mars.
So how do cells adapt to this potentially deadly environment? To prevent an exodus of water from the cell, halophiles offset the high salt in the environment by accumulating such compounds as potassium and glycine-betaine. This allows a balance of salts inside and outside of the cell preventing water from flowing outward as would be the case if lower salt levels existed within the cells.
ACIDITY AND ALKALINITY
Yellowstone National Park has bubbling acid hot-springs that would make a witch’s cauldron seem benign. They also teem with life. Once again we have been astounded that such environments harbor life.
Acidity and alkalinity are measures of the concentration of protons; the units used are pH units. The lower the number (down to zero), the higher the acidity. The higher (up to 14), the more alkaline. A neutral pH near 7 is optimal for many biological processes, although some — such as the light reactions of photosynthesis — depend on pH gradients. In nature, pH can be high, such as in soda lakes or drying ponds, or as low as 0 and below. Organisms that live at either extreme do this by maintaining the near-neutral pH of their cytoplasm (i.e. the liquid and materials within their cells).
Low pH is the realm of acidophiles — “acid lovers”. If you are looking for champion acid lovers, forget fish and cyanobacteria which have not been found below pH 4, or even plants and insects which don’t survive below pH 2 to 3. The extreme acidophiles are microbes. Several algae, such as the unicellular red alga Cyanidium caldarium and the green alga Dunaliella acidophila, are exceptional acidophiles both of which can live below pH 1. Three fungi, Acontium cylatium, Cephalosporium sp., and Trichosporon cerebriae, grow near pH 0. Another species, Ferroplasma acidarmanus, has been found growing at pH 0 in acid mine drainage in Iron Mountain in California. These polyextremophiles (tolerant to multiple environmental extremes) thrive in a brew of sulfuric acid and high levels of copper, arsenic, cadmium, and zinc with only a cell membrane and no cell wall.
HIGH TEMPERATURE
Temperature is a critical parameter because it determines whether liquid water is present. If temperature is too low, enzymatic activity slows, membrane fluidity decreases. Below freezing ice crystals form that slice through cell membranes. High temperatures can irreversibly alter the structure of biomolecules such as proteins, and increase membrane fluidity. The solubility of gasses in water is correlated with temperature, creating problems at high temperature for aquatic organisms requiring oxygen or carbon dioxide.
As it happens, organisms can outwit theory. Geysers, hotsprings, fumaroles and hydrothermal vents all house organisms living at or above the boiling point of water. The most hyperthermophilic (VERY hot loving) organisms are Archaea, with Pyrolobus fiimarii (of the Crenarchaeota), a nitrate-reducing chemolithotroph (an organism that derives energy from minerals), capable of growing at up to 113°C, is the current champion. As such, these hyperthermophiles are able to prevent the denaturation and chemical modification (breakdown) of DNA which normally occurs at or around a comparatively cool 70°C. The stability of nucleic acids is enhanced by the presence of salts which protect the DNA from being destroyed.
Thermophily (living in hot places) is more common than living in scalding, ultra-hot locales, and includes phototrophic bacteria (i.e., cyanobacteria, and purple and green bacteria who derive energy from photosynthesis), eubacteria (i.e., Bacillus, Clostridium, Thiobacillus, Desulfatomaculum, Thermos, lactic acid bacteria, actinomycetes, spirochetes, and numerous other genera), and the Archaea (i.e., Pyrococcus, Thermococcus, Thermoplasma, Sulfolobus, and the methanogens). In contrast, the upper limit for eukaryotes is about 60°C, a temperature suitable for some protozoa, algae, and fungi. The maximum temperature for mosses is another 10° lower, vascular plants (house plants, trees) about 48°C, and fish 40°C.
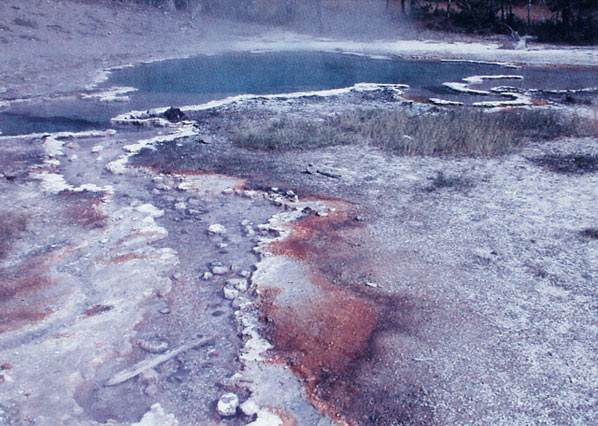
LOW TEMPERATURE
Representatives of all major forms of life inhabit temperatures just below 0°C. Think winter, think polar waters. While sperm banks and bacterial culture collections rely on the preservation of live samples in liquid nitrogen at -196°C, the lowest recorded temperature for active microbial communities and animals is substantially higher at -18°C.
Freezing of water located within a cell is almost invariably lethal. The only exception to this rule known from nature is the nematode Panagrolaimus davidi which can withstand freezing of all of its body water. In contrast, freezing of extracellular water — water outside of cells — is a survival strategy used by a small number of frogs, turtles and one snake to protect their cells during the winter. Survival of freezing must include mechanisms to survive thawing, such as the production of special proteins or “cryoprotectants” (additives that protect against the cold) called “antifreeze” proteins. The other method to survive freezing temperatures is to avoid freezing in the first place. Again “antifreeze” molecules are produced which can lower the freezing point of water 9 to 18°C. Fish in Antarctic seas manage to employ these mechanisms to their advantage.
Other changes with low temperature include changes in the structure of a cell’s proteins — most notably their enzymes — so as to allow them to function at lower temperatures. The fluidity of cell membranes decreases with temperature. In response, organisms that are able to adapt to cold environments simply increase the ratio of unsaturated to saturated fatty acids thus retaining the required flexibility of membranes.
RADIATION
Radiation is a hazard even on a comfortable planet like Earth. Sunlight can cause major damage unless mechanisms are in place to repair — or at least limit — the damage. Humans lacking the capacity to repair ultraviolet (UV) damage have xeroderma pigmentosa. This disease is so serious that suffers cannot leave their house during the day unless completely covered, and must even shade the windows in their homes.
Once you leave the protected surface of Earth, things can get more hostile. One of the major problems that organisms might face during interplanetary transfer (inside a rock blasted off of a planet by a large impact event for example), living on Mars, or even at high altitudes on Earth is the high levels of UV (ultraviolet) radiation.
In space there is cosmic and galactic radiation to contend with as well. The dangers of UV and ionizing radiation range from inhibition of photosynthesis up to damage to nucleic acids. Direct damage to DNA or indirect damage through the production of reactive oxygen molecules can alter the sequence or even break DNA strands.
Several bacteria including two Rubrobacter species and the green alga Dunaliella bardawil, can endure high levels of radiation. Deinococcus radiodurans, on the other hand, is a champ and can withstand up to 20 kGy of gamma radiation and up to 1,000 joules per square meter of UV radiation. Indeed, D. radiodurans can be exposed to levels of radiation that blow its genome into pieces only to have the organism repair its genome and be back to normal operations in a day.
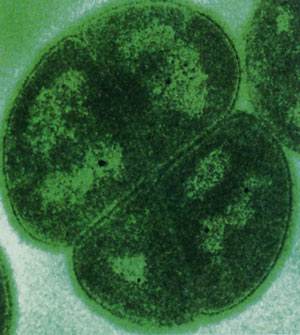
This extraordinary tolerance is accomplished through a unique repair mechanism which involves reassembling damaged (fragmented) DNA. Scientists at the Department of Energy are looking to augment the D. radiodurans genome such that it can be used to clean up mixed toxic and radioactive spills. So eager are biotechnologists to understand just how D. radiodurans does what it does that its genome was among the first organisms to be fully sequenced.
Gravity is a constant force in our lives; who has not imagined what it would be like to be an astronaut escaping gravity even temporarily? The universe offers a variety of gravitational experiences, from the near absence of gravity’s effects in space (more accurately referred to as microgravity) to the oppressive gravitational regimes of planets substantially larger than ours. Gravitational effects are more pronounced as the mass of an organism increases. That being said, flight experiments have revealed that even individual cells respond to changes in gravity. Cell cultures carried aboard various spacecraft including kidney cells and white blood cells showed marked alterations in their behavior, some of which is directly due to the absence of the effects of a strong gravity field. Indeed, recent work conducted aboard Space Shuttle missions has shown that there is a genetic component (as yet understood) to kidney cell responses to microgravity exposure.
PRESSURE
Pressure increases with depth, be it in a water column or in rock. Hydrostatic (water) pressure increases at a rate of about one-tenth of an atmosphere per meter depth, whereas lithostatic (rock) pressure increases at about twice that rate. Pressure decreases with altitude, so that by 10 km above sea level atmospheric pressure is almost a quarter of that at sea level.
The boiling point of water increases with pressure, so water at the bottom of the ocean remains liquid at 400°C. Because liquid water normally does not occur above about 100°C, increased pressure should increase the optimal temperature for microbial growth, but surprisingly pressure only extends temperature range by a few degrees suggesting that it is temperature itself that is the limiting factor.
The Marianas trench is the world’s deepest sea floor at 10,898 m, yet it harbors organisms that can grow at temperature and pressure we experience everyday. It has also yielded obligately piezophilic species (i.e. organisms that are pressure loving and can only grow under high pressure) that can only grow at the immense pressures found at the ocean’s greatest depths.
OTHER EXTREME CONDITIONS
A bit of creative thinking suggests other physical and chemical extremes not considered here, including unusual atmospheric compositions, redox potential, toxic or xenobiotic (manmade) compounds, and heavy metal concentration. There are even organisms such as Geobacter metallireducens that can survive immersion in high levels of organic solvents such as those found in toxic waste dumps. Others thrive inside the cooling water within nuclear reactors. While these organisms have received relatively little attention from the extremophile community, the search for life elsewhere may well rely on a better understanding of these extremes.
EXTREMOPHILES AND ASTROBIOLOGY
The study of extremophiles holds far more than Guinness Book of World Records-like fascination. Seemingly bizarre organisms are central to our understanding of where life may exist and where our own terrestrial life may one day travel. Did life on Earth originate in a hydrothermal vent? Will extremophiles be the pioneers that make Mars habitable for our own more parochial species?
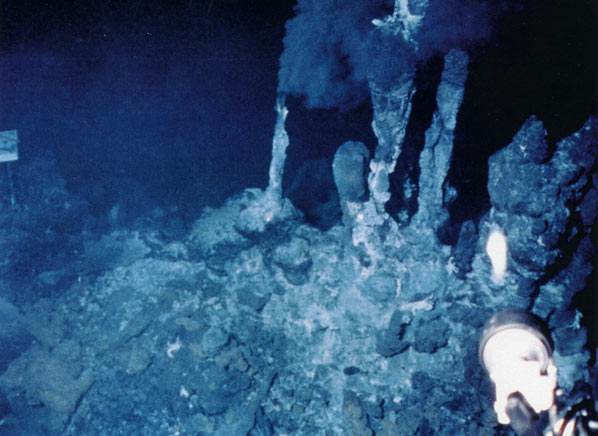
Happily, extremophile research has a lucrative side. Industrial processes and laboratory experiments may be far more efficient at extremes of temperature, salinity and pH, and so on. Natural products made in response to high levels of radiation or salt have been sold commercially. Glory too goes to those working with extremophiles. At least one Nobel Prize, that for the invention of the polymerase chain reaction (PCR), would not have been possible without an enzyme from a thermophile. As the world of molecular biology has become increasingly reliant on products from extremophiles, they will continue be the silent partner in future awards.
Current work on extremophiles in space focuses on four major environments: manned-flight vehicles, interplanetary space (because of the potential for panspermia), Mars and Europa because of the possibility of liquid water — and thus life.
MARS: HABITABLE?
Mars is, at first blush, inhospitable. Temperatures are, for the most part, frigid, exposure to ultraviolet radiation is high, and the surface is highly oxidizing, precluding the presence of organic compounds on the surface. The atmospheric pressure is very low (similar to that of Earth’s uppermost atmosphere) so liquid water is unstable on the surface. Yet hydrogeological evidence from Mars Global Surveyor hints that liquid water may even flow today under the surface. Previous evidence seems to show that it once flowed much more freely on the surface in ancient times.
Could Mars harbor subsurface life, similar to the subsurface or hydrothermal communities found on Earth? If so, it would be protected from surface radiation, damaging oxidants, and have access to liquid water. Mars is rich in carbon dioxide, the raw material used by plants to produce organic carbon. Life has been found at the depths of Earth’s oceans and several kilometers below the surface inside of rocks. If it did arise during a warmer, wetter period in Mars’ history, perhaps it managed to migrate into warmer, more clement regions of the planet’s interior before the surface became uninhabitable.
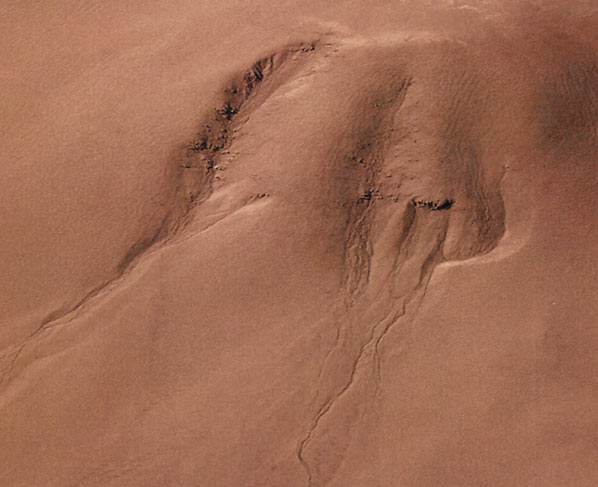
THE LARGE MOONS OF JUPITER: UNDERGROUND OCEANS
With evidence mounting that one or more of the large moons of Jupiter (Europa, Ganymede, Callisto) have ice-covered oceans, the possibility of life on these moons becomes a subject of scientific discourse. One of these, Europa, has an ice layer too thick to allow enough light to get through to allow photosynthesis, the process that drives much of terrestrial life including those under the perennially ice-covered lakes of Antarctica. However, Chris Chyba from the SETI Institute has suggested that chemistry in the ocean’s ice cover, driven by charged particles accelerated in Jupiter’s magnetosphere, could produce sufficient organic and oxidant molecules for a Europan biosphere to be sustained. The Galileo spacecraft has detected a weak magnetic field on Callisto, suggesting that salt water may lie beneath an ice-covered surface. Supportive evidence exists as well for an ocean with Ganymede. Several of Saturn’s moons and other outer solar system bodies may also hold the potential for having a subsurface ocean.
NAKED IN SPACE: THE ULTIMATE EXPOSURE
Panspermia (“seeds spread far”), the idea that life can travel through space from one hospitable location to the another, is no longer wild speculation. Space is extremely cold, subject to unfiltered solar radiation, solar wind, galactic radiation, space vacuum, and to negligible gravity. But this treacherous realm can be crossed by life.
We know from Mars meteorites such as the (now) famous ALH84001 sample that a natural vehicle exists for interplanetary transport. These meteorites contain organic compounds from Mars, showing that such compounds can survive the journey. Moreover, studies have shown that given a rock of sufficient size, conditions within a rock thrown off of Mars — and then later entering Earth’s atmosphere — can remain cool enough such that not just organic material, but also microbes contained within, could (theoretically) survive the trip.
The criticism that life cannot endure extended periods in space is now being tested experimentally in space simulation facilities in the U.S. and Germany, and through unmanned flight experiments. NASA’s Long Duration Exposure Facility and the European Space Agency’s BioPan space experiments showed that microbes can survive direct exposure to the raw conditions of space. Survivors to date include spores of Bacillus subtilis and halophiles in the active (vegetative) state. Hopes for further experiments of this nature rest on both unmanned flights and the ESA Exposed Facility planned for the International Space Station.
SUMMARY
Earth provides us with a wondrous array of life’s adaptations. Indeed, by studying the extremophiles here on Earth, we may get the first clear indication of what ET could be like — or at least the range of things they might eat and breathe.
References:
Norton, C. F. & Grant, W. D. Survival of halobacteria within fluid inclusions in salt crystals. J. Gen. Microbial., 134, 1365-73 (1988).
Rothschild, L. J., Giver, L. J., White, M. R. & Mancinelli, R. L. Metabolic activity of microorganisms in gypsum-halite crusts. J. Phycol., 30:431-438 (1994).
Vreeland, R. H., Rosenzweig, W. D. & Powers, D. W. Isolation of a 250 millionyear-old halotolerant bacterium from a primary salt crystal. Nature 407, 897- 900 (2000).
Macelroy, R. D. Some comments on the evolution of extremophiles. Biosystems 6, 74-75 (1974).
Reviews:
Rothschild, L. J. & Mancinelli, R. L. Life in Extreme Environments. Nature 409: 1092-1101 (2001).
Mancinelli, R.L. & Li. Rothschild. “Extremophiles: Who, What, Where and How.” McMillan Encyclopedia of Biology, 2002. In press.
Madigan. M. T. & Marrs, B. L. Extremophiles. Scientific Am. 276(4), 82-87 (1997)
Horikoshi, K. & Grant, W. D. Extremophiles. Microbial Life in Extreme Environments. (Wiley-Liss, New York 1998).
Seckbach, J., (ed.) Journey to Diverse Microbial Worlds: Adaptation to Exotic Environments. (Kluwer Academic Publishers, Dordrecht 2000).